From Bioblast
News and Events | Working Groups | Short-Term Scientific Missions | Management Committee | Members |
COST Action CA15203 (2016-2021): MitoEAGLE
Evolution-Age-Gender-Lifestyle-Environment: mitochondrial fitness mapping
The protonmotive force and respiratory control
File:OXPHOS system.jpg
Fig. 1. The mitochondrial respiratory system. In oxidative phosphorylation the electron transfer system (A) is coupled to the phosphorylation system (B). See Eqs. 4 and 5 for further explanation.
Mitochondrial respiratory control: a conceptual perspective on coupling states in mitochondrial preparations
- MITOEAGLE recommendations Part 1.
- » Work in progress: Download last update (Version 17) 2017-08-17:
- » Versions
- » Work in progress: Download last update (Version 17) 2017-08-17:
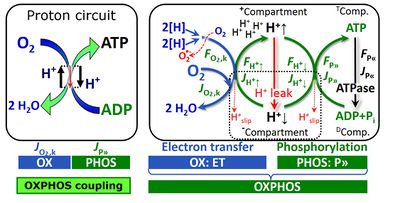
Fig. 2. The proton circuit and coupling in oxidative phosphorylation (OXPHOS). Modified after Gnaiger 2014 MitoPathways.
- MITOEAGLE Terminology Group
- First draft, updates, and corresponding author: E. Gnaiger
- Contributing co-authors: Confirming to have read the final manuscript, possibly to have made additions or suggestions for improvement, and to agree to implement the recommendations into future manuscripts, presentations and teaching materials. (alphabetical, to be extended):
- M.G. Alves, D. Ben-Shachar, G.C. Brown, G.R. Buettner, P.M. Coen, J.L. Collins, L. Crisostomo, M.S. Davis, C. Doerrier, E. Elmer, A. Filipovska, P.M. Garcia-Roves, D.K. Harrison, K.T. Hellgren, C.L. Hoppel, J. Iglesias-Gonzalez, P. Jansen-Dürr, B.H. Goodpaster, B.A. Irving, S. Iyer, T. Komlodi, V. Laner, H.K. Lee, A.T. Meszaros, N. Moisoi, A. Molina, A.L. Moore, J. Neuzil, R.K. Porter, K. Nozickova, P.J. Oliveira, K. Renner-Sattler, J. Rohlena, L.A. Sazanov, O. Sobotka, R. Stocker, I. Szabo, M. Tanaka, L. Tretter, B. Velika, A.E. Vercesi, Y.H. Wei
- MITOEAGLE Terminology Group
- Supporting co-authors: Confirming to have read the final manuscript, and to agree to implement the recommendations into future manuscripts, presentations and teaching materials. (alphabetical, to be extended):
- R.A. Brown, A.J. Chicco, T. Dias, G. Distefano, H. Dubouchaud, L.F. Garcia-Souza, G. Keppner, A. Krajcova, M. Markova, D. O'Gorman, C.M. Palmeira, P.X. Petit, K. Siewiera, P. Stankova, Z. Sumbalova
- Journal: Int J Biochem Cell Biol (as discussed at MITOEAGLE Barcelona 2017); Open Access is a requirement. Final decision has to be made.
New Fig. 6 and text in Version 16
- 2018-08-16 Erich Gnaiger - added Fig. 6 and text in Version 16:
- Fig. 6 summarizes the three coupling states, ETS, LEAK and OXPHOS, and puts them into a schematic context with the corresponding respiratory rates, abbreviated as E, L and P, respectively. This clarifies that E may exceed or be equal to P, but E cannot theoretically be lower than P. E<P must be discounted as an artefact, which may be caused experimentally by (i) using high and inhibitory uncoupler concentrations (Gnaiger 2008), (ii) non-saturating [ADP] or [Pi] (State 3), (iii) high oligomycin concentrations applied for measurement of L before titrations of uncoupler, when oligomycin exerts an inhibitory effect on E, or (iv) loss of oxidative capacity during the time course of the respirometric assay with E measured subsequently to P (Gnaiger 2014). On the other hand, E>P is observed in many types of mitochondria and depends on (i) the excess ETS capacity pushing the phosphorylation system (Fig. 1B) to the limit of its capacity of utilizing ∆pmt, (ii) the pathway control state with single or multiple electron input into the Q-junction and involvement of three or less coupling sites determining the H+out/O2 coupling stoichiometry (Fig. 2A), and (iii) the biochemical coupling efficiency expressed as (E-L)/E, since any increase of L causes an increase of P upwards to the limit of E. The excess E-P capacity, ExP=E-P, therefore, provides a sensitive diagnostic indicator of specific injuries of the phosphorylation system, when E remains constant but P declines relative to controls (Fig. 6). Substrate cocktails supporting simultaneous convergent electron transfer to the Q-junction for reconstitution of TCA cycle function stimulate ETS capacity, and consequently increase the sensitivity of the ExP assay.
- ... In general, it is inappropriate to use the term ATP production for the difference of oxygen consumption measured in states P and L. The difference P-L is the upper limit of the part of OXPHOS capacity which is free (corrected for LEAK respiration) and is fully coupled to phosphorylation with a maximum mechanistic stoichoimetry, ≈P = P-L (Fig. 6).
Perspective added in Version 17
- To provide an overall perspective of mitochondrial physiology we may link cellular bioenergetics to systemic human respiratory activity, without yet addressing cell- and tissue-specific mitochondrial function. A routine O2 consumption of 3.3 nmol∙s-1∙g-1 body mass corresponds to -110 W catabolic energy flow at a body mass of 70 kg and -470 kJ/mol O2. Considering a cell count of 514∙106 cells per g tissue mass and an estimate of 300 mitochondria per cell (Ahluwalia 2017), the average oxygen flow per million cells is 97 pmol∙s-1∙10-6 at Jm,O2peak of 50 nmol·s-1·g-1 (compare experimental results on cultured cells given above). We can define us as the sum of 36∙1012 cells (36 trillion cells). Mitochondrial fitness of our 11∙1015 mitochondria (11 quadrillion mt) is indicated if O2 flow of 0.0003 pmol∙s-1∙mt-1 at rest can be activated to 0.005 pmol∙s-1∙mt-1 at maximum ergometric performance.
- Scope
- Target a broad audience – introduce the new generation of investigators.
- List of terms including historical terms; abbreviations (mtDNA, mt to abbreviate mitochondr*); OXPHOS capacity versus State 3 (discuss saturating ADP/Pi .. concentrations).
- Protonmotive force: not a force defined in physics, but an isomorphic force of statistical and nonequilibrium thermodynamics.
- Flux and flow: clarification of normalization.
- Scientific terminology should be general and platform-independent, meeting the demands of all working groups.
- Scope
Abstract
- Clarity of concepts and consistency of nomenclature is a hallmark of the quality of a research field across its specializations, aimed at facilitating transdisciplinary communication and education. As mitochondrial physiology continues to expand, the necessity for improved harmonization of nomenclature on mitochondrial respiratory states and rates has become apparent. Peter Mitchell’s protonmotive force across the inner mitochondrial membrane, Δpmt, establishes the link between electron transfer and phosphorylation of ADP to ATP, and between the electric and chemical components of energy transformation (ΔΨmt and ΔpH). This unifying concept provides the framework for developing a consistent terminology on mitochondrial physiology and bioenergetics. We follow IUPAC guidelines on general terms of physical chemistry, extended by concepts of nonequilibrium thermodynamics and open systems. The nomenclature of respiratory sates in classical bioenergetics (States 1 to 5 in an experimental protocol) is incorporated into a concept-driven constructive terminology to address the meaning of each respiratory state and to focus primarily on the conceptual ‘why’ with clarification of the experimental ‘how’. LEAK states are evaluated to study arrested respiration, when oxygen consumption compensates mainly for the proton leak. OXPHOS capacity is measured at saturating concentrations of ADP and inorganic phosphate to obtain kinetic reference values for diagnostic applications. The oxidative capacity of the electron transfer system is determined in the ETS state, revealing the limitation of OXPHOS capacity mediated by the capacity of the phosphorylation system. Development of databases of mitochondrial respiratory control requires the application of strictly defined terms for comparison of respiratory states.
- ‘Every professional group develops its own technical jargon for talking about matters of critical concern. .. People who know a word can share that idea with other members of their group, and a shared vocabulary is part of the glue that holds people together and allows them to create a shared culture’ (Miller 1991).
Classical terminology for isolated mitochondria
Three fundamental coupling states of mitochondrial preparations and residual oxygen consumption
States and rates
- Force, Ftr: A generalized force (intensive quantity) in thermodynamics or ergodynamics is the partial Gibbs (Helmholtz) energy change per advancement of a transformation (tr).
- External flow, Iext
- Internal flow, Iint
- Open system
- Respiratory state
Normalization: fluxes and flows
- Units (important for a database); analogous to electic terms: Flow [C.s-1]; Flux [C.s-1.m-2]; Rate (?)
- Extensive quantity
- Oxygen flow, IO2
- Oxygen flux, JO2
- Mitochondrial marker
- Specific quantity
- Intensive quantity
List of selected terms and symbols
- Coupled respiration
- Dyscoupled respiration
- Isolated mitochondria, imt
- Mitochondria, mt (Greek mitos: thread; chondros: granule) are small structures within cells, which function in cell respiration as powerhouses or batteries. Mitochondria belong to the bioblasts of Richard Altmann (1894). Abbreviation: mt, as generally used in mtDNA. Singular: mitochondrion (bioblast); plural: mitochondria (bioblasts).
- Mitochondrial inner membrane, mt-im
- Mitochondrial outer membrane, mt-om
- Mitochondrial matrix, mt-matrix
- Mitochondrial membrane potential, mtMP, Δψmt
- Mitochondrial preparations, mtprep are isolated mitochondria (imt), tissue homogenate (thom), mechanically or chemically permeabilized tissue (permeabilized fibres, pfi) or permeabilized cells (pce). In these preparations the cell membranes are either removed (imt and smtp) or mechanically (thom) and chemically permeabilized (pfi), while the mitochondrial functional integrity and to a large extent the mt-structure is maintained.
- Mitochondrial respiration
- Noncoupled respiration
- Oligomycin, Omy
- Permeabilized tissue or cells, pti, pce
- Phosphorylation system
- Proton leak
- Proton pump
- Proton slip
- Protonmotive force, Δpmt
- Tissue homogenate, thom
- Uncoupler, U
Mitochondrial respiratory control: pathway states in mt-preparations
- MITOEAGLE recommendations Part 2.
- ‘It is essential to define both the substrate and ADP levels in order to identify the steady-state condition of the mitochondria during the experiment’ (Chance and Williams, 1956).
- The mitochondrial respiratory system
- Substrates and inhibitors
- Switch to pathway-related nomenclature instead of enzyme-linked terminology (N/NS/S versus CI/CI+II/CII)
Mitochondrial respiratory control: cell respiration
- MITOEAGLE recommendations Part 3.
- Intact cells versus mitochondrial preparations
- Intact cells, ce
- Basal respiration
- Cell respiration
- Resting metabolic rate
- ROUTINE state, state R: ROUTINE respiration of intact, viable cells is regulated according to physiological activity, at intracellular non-saturating ADP levels. R increases under various conditions of activation. When incubated in culture medium, cells maintain a ROUTINE level of activity, R (ROUTINE mitochondrial respiration; corrected for residual oxygen consumption due to oxidative side reactions). ROUTINE activity may include aerobic energy requirements for cell growth and is thus fundamentally different from the definition of basal metabolic rate (BMR). When incubated for short experimental periods in a medium devoid of fuel substrates, the cells respire solely on endogenous substrates at the corresponding state of ROUTINE activity, eR (e, endogenous substrate supply).
- Intact cells versus mitochondrial preparations
- It is difficult to stimulate living cells to maximum OXPHOS activity, since ADP and inorganic phosphate do not equilibrate across intact plasma membranes, and thus saturating concentrations of these metabolites can hardly be achieved in living cells. LEAK and ETS states, however, can be induced in viable cells with application of inhibitors of the phosphorylation system and uncouplers, respectively, due to the fact that cell membranes are highy permeable for these substances. External fuel substrates are taken up by living cells to various extents, and intracellular metabolism of exogenous and endogenous substrates supports mitochondrial respiration with a physiological substrate supply. In contrast, mt-preparations depend on the external supply of fuel substrates which support the electron transfer system with reducing equivalents. ETS competence of external substrates is required for all coupling states of mt-preparations (L, P, E) and depends on (i) transport of substrates across the inner mt-membrane or oxidation by dehydrogenases located on the outer face of the inner mt-membrane (e.g. glycerophosphate dehydrogenase complex, CGpDH), (ii) oxidation in the mt-matrix (TCA cycle dehydrogenases and other matrix dehydrogenases, e.g. mtGDH) or on the inner face of the inner mt-membrane (succinate dehydrogenase, CII), (iii) oxidation of substrates without accumulation of inhibitory endproducts (e.g. oxaloacetate inhibiting succinate dehydrogenase; NADH and oxaloacetate inhibiting malate dehydrogenase), and (iv) electron transfer through the membrane-bound ETS (mETS). Endproducts must be either easily exported from the matrix across the inner mt-membrane (e.g. malate formed from succinate via fumarate), or metabolized in the TCA cycle (e.g. malate-derived oxaloacetate forming citrate in the presence of external pyruvate&malate).
Mitochondrial respiratory control: coupling control ratios and control factors
- MITOEAGLE recommendations Part 4.
- ETS coupling efficiency, j≈E: j≈E = ≈E/E = (E-L)/E = 1-L/E
- LEAK control ratio, L/E
- Excess E-P capacity factor, jExP: jExp = (E-P)/E = 1-P/E
- OXPHOS control ratio, P/E
- OXPHOS coupling efficiency, j≈P: j≈P = ≈P/P = (P-L)/P = 1-L/P
- L/P coupling control ratio, L/P
- Respiratory acceptor control ratio, RCR: RCR = State 4/State 3
- netOXPHOS control ratio, ≈P/E: ≈P/E = (P-L)/E
- Uncoupling control ratio, UCR: UCR = E/R
Action
- » WG1 Action - WG1 MITOEAGLE protocols, terminology, documentation: Standard operating procedures and user requirement document: Protocols, terminology, documentation
- » WG1 Project application
- » 2017-07 MiPschool Obergurgl 2017
- » 2017-03 MITOEAGLE Barcelona 2017
- » 2016-11 MITOEAGLE 2016 Verona IT