From Bioblast
News and Events | Working Groups | Short-Term Scientific Missions | Management Committee | Members |
COST Action CA15203 (2016-2021): MitoEAGLE
Evolution-Age-Gender-Lifestyle-Environment: mitochondrial fitness mapping
The protonmotive force and respiratory control
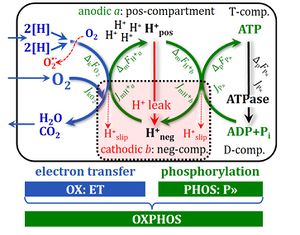
OXPHOS-coupled energy cycles. From Gnaiger 2014 MitoPathways.
- Scope of MITOEAGLE publications: respiratory control
- Target a broad audience – also the new generation
- List of terms including historical terms; abbreviations (mtDNA, mt to abbreviate mitochondr*); OXPHOS capacity versus State 3 (discuss saturating ADP/Pi .. concentrations)
- Scientific terminology should be general and platform independent - meet the demands of all working groups.
- Scope of MITOEAGLE publications: respiratory control
Mitochondrial respiratory control: a conceptual perspective on coupling states
- MITOEAGLE recommendations Part 1.
- MITOEAGLE Terminology Committee - first draft prepared by Erich Gnaiger (work in progress since 2017-04-18; last update 2017-04-24)
- Journal: Int J Biochem Cell Biol (as discussed at MITOEAGLE Barcelona 2017); Open Access is a requirement.
Abstract
- Clarity of concepts and consistency of nomenclature is a signature of the quality of a research area across its specializations, aimed at facilitating translational communication and teaching. The expanding field of mitochondrial respiratory physiology can benefit from the harmonization of nomenclature on mitochondrial respiratory states and control parameters. Peter Mitchell’s protonmotive, chemiosmotic force across the inner mitochondrial membrane, Δpmt, establishes the link between electron transfer and phosphorylation of ADP to ATP, and between the chemical (pH difference, ΔpH) and electric (mt-membrane potential difference, ΔΨmt) components of energy transformation. This unifying concept provides the framework upon which a consistent terminology on mitochondrial physiology and bioenergetics can be based. IUPAC guidelines are followed for general terms of physical chemistry, extended by concepts of nonequilibrium thermodynamics and open systems. The differential nomenclature of classical bioenergetics (numerical differentiation of experimental protocol-linked respiratory States 1, 2, 3, 4 and 5) is incorporated into a concept-driven constructive terminology to address the basic meaning of a respiratory state and direct attention from the experimental ‘how’ to the conceptual ‘why’. LEAK states are evaluated to study resting respiration, L, compensating mainly for the proton leak. OXPHOS capacity, P, is measured at saturating levels of ADP and inorganic phosphate to obtain kinetic reference values for diagnostic applications. The ETS state differentiates the oxidative capacity of the electron transfer system, E, from OXPHOS capacity, revealing the limitation of P by the phosphorylation system. Development of databases on mitochondrial respiratory control requires application of strictly defined terms for comparison of respiratory states.
Introduction
- Every study of mitochondrial function and disease in human tissues and cells is faced with evolution, age, gender, lifestyle and environment (EAGLE) as essential background conditions characterizing the individual patient, subject, study group, species, tissue or – to some extent - cell line. This range of factors is too wide to be accommodated in any single project on mitochondrial respiration. Only a large and well coordinated network can manage to generate the necessary type, quality and number of consistent data to address the complexity of EAGLE. The global MITOEAGLE network is a strategic innovation to develop harmonization protocols towards generating a rigorously monitored database on mitochondrial respiratory function. A quality control and data management system (QMS) is necessary to interrelate results obtained in a large number of studies, to interpret pathological phenotypes, and to set results into the multidimensional context of EAGLE.
- Reliability and comparability of quantitative results depends on accuracy of measurement under well defined conditions. To relate results of studies carried out by different research groups further requires reporting in a conceptually meaningful framework, assigning values expressed in common units (the measurements) to specific attributes (the rows in a table). If the attribute is well defined and understood (‘well defined experimental conditions’), it does not matter which names or symbols are given to the rows in the table of a database. But if terminology is vague or ambiguous when characterizing the type of result, then comparison of even the most accurate data can lead to confusion and may turn valuable signals into wasteful noise. Clarification of some fundamental terms used in studies of mitochondrial respiratory control, therefore, represents a basic component of a QMS. Even if standardization of nomenclature remains a goal of the optimist and is out of reach in the real world, harmonization of the technical jargon will improve the awareness of the intricate meaning of divergent scientific vocabulary. The MITOEAGLE Terminology Committee aims at accomplishing the ambitious goal to harmonize, unify and thus simplify the terminology in the field of mitochondrial physiology.
Respiratory coupling states: from bioenergetics to mitochondrial physiology
- ‘Every professional group develops its own technical jargon for talking about matters of critical concern. .. People who know a word can share that idea with other members of their group, and a shared vocabulary is part of the glue that holds people together and allows them to create a shared culture’ (Miller 1991).
Constructive versus differential terminologies
- Five classical states of mitochondrial respiration and cytochrome redox states have been introduced by Chance and Williams (1955; 1956). These respiratory states are explained by a protocol with isolated mitochondria (imt) in a closed respirometric chamber:
- ‘It is essential to define both the substrate and ADP levels in order to identify the steady-state condition of the mitochondria during the experiment’ (Chance and Williams, 1956).
- State 1 is obtained after addition of imt to isotonic respiration medium containing inorganic phosphate but no adenylates, specifically no ADP, and no fuel substrates (CHNO).
- State 2 is induced by addition of a high level of ADP, which stimulates transiently respiration on the basis of endogenous fuel substrates, followed by a low respiratory activity limited by fuel substrate availability.
- State 3: Respiratory fuel substrates are added to initiate State 3. Respiration is stimulated while the ADP level is still high and supports coupled energy transformation in oxidative phosphorylation.
- State 4: If the imt preparation is of high quality, devoid of ATPase activity and well coupled, gradual depletion of ADP by phosphorylation to accumulating ATP leads to a sudden decline of oxygen uptake in the transition from State 3 to State 4, when a maximum mt-membrane potential and ATP/ADP ratio are maintained. State 4 respiration is limited by physiological uncoupling (mainly the intrinsic proton leak). A repeated titration of high ADP can restore active respiration in State 3.
- State 5 is the anoxic state after the aerobic-anoxic transition, with zero respiration in a closed respirometric system. Ideal closed respirometers without any backdiffusion of oxygen into the chamber are difficult to obtain in practice, whence this steady state (State 5) is characterized by the oxygen-limited respiration compensating for the oxygen backdiffusion at near-zero oxygen levels.
- It has been suggested to extend the differential nomenclature (States 1 to 5) by a concept-driven constructive terminology on coupling states (Gnaiger 2009). In a constructive theory, the meanings are expressed by terms which carry information on the concept they represent (Miller 1991). The concept-driven terminology must be (i) general and not restricted to a particular experimental protocol with imt in a closed oxygraph, (ii) applicable to any mt-preparation, (iii) describe and guide studies with any experimental platform using a wide variety of experimental protocols, and (iv) extend the focus of classical bioenergetics on coupling mechanisms and coupling sites of oxidative phosphorylation to perspectives on mitochondrial physiology and pathology with diagnostic applications. This does not lead simply to a system of synonyms, but to a conceptual differentiation between the classical respiratory states which have been entirely sufficient to describe a specific bioenergetic protocol on one hand, and the extended constructive framework of the theory of mitochondrial physiology on the other hand.
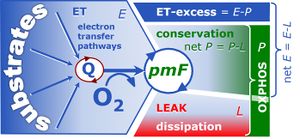
Capacities of the electron transfer system, oxidative phosphorylation and resting LEAK respiration (ETS, OXPHOS, LEAK) and four-compartmental OXPHOS model. (i) Capacity of the ETS module, E, in the noncoupled state, generating the protonmotive force, Δpmt. OXPHOS capacity, P, is partitioned into (ii) the dissipative LEAK component, L (disspation of Δpmt), and (iii) the free OXPHOS capacity, ≈P=P-L (energy conversion driven by Δpmt). If ≈P is limited by the capacity of the phosphorylation system, then (iv) the apparent ETS excess capacity, ExP=E-P, is available to drive coupled processes other than phosphorylation without competing with ATP production. Free divided by total ETS capacity, ≈E/E, is the ETS coupling efficiency. Free divided by total OXPHOS capacity, ≈P/P, is the OXPHOS coupling efficiency. From Gnaiger 2014 MitoPathways.
Three fundamental coupling states of mitochondrial preparations
- ETS, state E: Noncoupled state at optimal uncoupler concentration for maximum oxygen flux as a measure of electron transfer system (ETS) capacity, in the presence of fuel substrates and oxygen. The abbreviation State 3u is used frequently in bioenergetics, to indicate the state of maximum respiration, E, without sufficient emphasis on the fundamental difference between state P (OXPHOS capacity; coupled, with an uncoupled component) and state E (ETS capacity, noncoupled).
- OXPHOS, state P: In mitochondrial physiology and pathology, maximal mitochondrial respiration in the coupled state is measured for quantitative determination of OXPHOS capacity, P (Gnaiger 2009). State P is the ADP-activated state at maximum oxygen flux as a measure of the capacity for oxidative phosphorylation (OXPHOS), supported by saturating ADP and inorganic phosphate concentrations and in the presence of fuel substrates and oxygen. The definition of State 3 lacks a fundamental attribute of OXPHOS capacity. 'High ADP' in State 3 is a concentration of ADP specifically selected to allow measurememt of a State 3 to State 4 transition of isolated mitochondria in a closed respirometric system. Starting at oxygen levels near air saturation, the 'high ADP' concentration added must be low enough to allow phosphorylation to ATP at a coupled oxygen consumption that does not lead to oxygen depletion during the transition to State 4. In contrast, OXPHOS capacity requires evaluation of kinetically saturating ADP concentrations, which are usually an order of magnitude higher than 'high ADP' in State 3.
- LEAK, state L: Resting state of non-phosphorylating respiration when oxygen flux is maintained mainly to compensate for the proton leak in the absence of ATP synthesis, either due to absence or depletion of ADP at a maximum ATP/ADP ratio, or due to inhibition of phosphorylation by inhibitor such as oligomycin or atractyloside, in the presence of fuel substrates and oxygen. State 4 represents an overestimation of LEAK respiration if ATPase activity prevents final accumulation of ATP and maintains a continuous stimulation of respiration by recycled ADP. This can be tested by inhibition of phosphorylation (oligomycin).
- ROX: Residual oxygen consumption (ROX) is not characteristic of a coupling state, but represents a baseline for correction of mitochondrial respiration in the presence of oxygen and the absence of fuel substrates or after addition of inhibitors of the electron transfer system. Total respiration is thus partitioned into a component functionally linked to the electron transfer system and a component not linked to the ETS (ROX). ROX is frequently considered to be equivalent to non-mitochondrial respiration, which ignores the fact that mitochondria contain enzymes which catalyse oxygen-consuming reactions not linked to the ETS and OXPHOS.
- In kinetic studies an infinite number of intermediary steady states can be established (i) between LEAK an OXPHOS, using ADP-regenerating enzyme systems or steady-state ADP injections, (ii) between LEAK and ETS or OXPHOS and ETS, by uncloupler titrations, and (iii) between any of the three fundamental coupling states and ROX, by inhibitor titrations.
Intact cells versus mitochondrial preparations
- ROUTINE state, state R: ROUTINE respiration of intact, viable cells is regulated according to physiological activity, at intracellular non-saturating ADP levels. R increases under various conditions of activation. When incubated in culture medium, cells maintain a ROUTINE level of activity, R (ROUTINE mitochondrial respiration; corrected for residual oxygen consumption due to oxidative side reactions). ROUTINE activity may include aerobic energy requirements for cell growth and is thus fundamentally different from the definition of basal metabolic rate (BMR). When incubated for short experimental periods in a medium devoid of fuel substrates, the cells respire solely on endogenous substrates at the corresponding state of ROUTINE activity, eR (e, endogenous substrate supply).
- It is difficult to stimulate living cells to maximum OXPHOS activity, since ADP and inorganic phosphate do not equilibrate across intact plasma membranes, and thus saturating concentrations of these metabolites can hardly be achieved in living cells. LEAK and ETS states, however, can be induced in viable cells with application of inhibitors of the phosphorylation system and uncouplers, respectively, due to the fact that cell membranes are highy permeable for these substances. External fuel substrates are taken up by living cells to various extents, and intracellular metabolism of exogenous and endogenous substrates supports mitochondrial respiration with a physiological substrate supply. In contrast, mt-preparations depend on the external supply of fuel substrates which support the electron transfer system with reducing equivalents. ETS competence of external substrates is required for all coupling states of mt-preparations (L, P, E) and depends on (i) transport of substrates across the inner mt-membrane or oxidation by dehydrogenases located on the outer face of the inner mt-membrane (e.g. glycerophosphate dehydrogenase complex, CGpDH), (ii) oxidation in the mt-matrix (TCA cycle dehydrogenases and other matrix dehydrogenases, e.g. mtGDH) or on the inner face of the inner mt-membrane (succinate dehydrogenase, CII), (iii) oxidation of substrates without accumulation of inhibitory endproducts (e.g. oxaloacetate inhibiting succinate dehydrogenase; NADH and oxaloacetate inhibiting malate dehydrogenase), and (iv) electron transfer through the membrane-bound ETS (mETS). Endproducts must be either easily exported from the matrix across the inner mt-membrane (e.g. malate formed from succinate via fumarate), or metabolized in the TCA cycle (e.g. malate-derived oxaloacetate forming citrate in the presence of external pyruvate&malate).
States and rates
- Metabolic fluxes and steady-state variables are measured in defined mitochondrial respiratory states. Strictly, steady states can be obtained only in open systems, in which internal transformations (e.g. oxygen consumption or enthalpy change of chemical reactions) are instantaneously compensated by external flows (oxygen supply or heat flow across the system boundaries), such that changes do not occur in the system but only in the environment. Mitochondrial respiratory states measured in closed systems may satisfy the criteria of pseudo-steady states for limited periods of time, when the changes occurring in the system (e.g. oxygen concentration; ADP concentration; mt-membrane potential) do not exert significant effects on the metabolic fluxes (respiration, phosphorylation of ADP to ATP). Such pseudo-steady states require saturation levels of substrates to be maintained and thus depend on the kinetics of the processes under investigation.
- Force, Ftr: A generalized force (intensive quantity) in thermodynamics or ergodynamics is the partial Gibbs (Helmholtz) energy change per advancement of a transformation (tr).
- Intensive quantity
- Internal flow, Iint
- Open system
- Oxygen flow, IO2
- Oxygen flux, JO2
- Respiratory state
Normalization: fluxes and flows
- Units (important for a database); analogous to electic terms: Flow [C.s-1]; Flux [C.s-1.m-2]; Rate (?)
- Extensive quantity
- External flow, Iext
- Mitochondrial marker
- Specific quantity
Partitioning of respiration and respiratory coupling control ratios
- Gnaiger 2014 MitoPathways
- LEAK respiration in the OXPHOS state as discussed below provides an example for the general fact that partitioning of respiration by subtracting oxygen consumption measured separately in different coupling states must be critically evaluated. Due to the simplicity of the approach, however, such partitioning has become widely applied and may be used for comparative purposes, particularly in the context of respiratory control ratios and control factors, contrasting different experimental groups and various tissues and species.
- Gnaiger 2014 MitoPathways
Partitioning of respiration within a respiratory coupling control state
- Partitioning of LEAK respiration: LEAK respiration can be partitioned into patial oxygen consumption compensating for (1) proton leaks, (2) proton slip, (3) cation cycling, and (4) electron leak.
- LEAK respiration in the OXPHOS state, LP: Since OXPHOS is partially coupled, intrinsic uncoupling and dyscoupling contribute to the control of flux in the OXPHOS state. Oxygen consumption in the OXPHOS state, therefore, is partitioned into the free OXPHOS capacity, ≈P, and nonphosphorylating LEAK respiration, LP. It is frequently assumed that LEAK respiration, L, as measured in the LEAK state, overestimates the LEAK component of respiration, LP, as measured in the OXPHOS state, particularly if the protonmotive force is not adjusted to equivalent levels in L and LP. However, if the LEAK component increases with enzyme turnover during P, the low enzyme turnover during L may counteract the effect of the higher Δpmt (Garlid et al 1993).
- P = ≈P + LP.
Partitioning by subtraction of respiration in different coupling states
- Free OXPHOS capacity, ≈P: ≈P=P-L is the partial oxygen consumption which is strictly coupled to phosphorylation of ADP to ATP, ~P, when partitioning OXPHOS capacity into a coupled and uncoupled component, P=≈P + L.
- Free ETS capacity, ≈E: ≈E is the ETS capacity corrected for LEAK respiration, ≈E = E-L. ≈E is the respiratory capacity potentially available for phosphorylation of ADP to ATP and ion transport. Oxygen consumption in the ETS state, therefore, is partitioned into the free ETS capacity, ≈E, and LEAK respiration, L, compensating for proton leaks, slip and cation cycling, E = ≈E + L.
- Excess E-P capacity, ExP: ExP is the difference of the ETS capacity and OXPHOS capacity, ExP = E-P. At ExP > 0, the capacity of the phosphorylation system exerts a limiting effect on OXPHOS capacity. In addition, ExP depends on coupling efficiency, since P aproaches E at increasing uncoupling. When partitioning ETS capacity, a OXPHOS-linked component (intrinsig LEAK respiration plus strictly coupled respiration) is distinguished from ExP as the apparent excess capacity above the OXPHOS-linked component.
Respiratory coupling control ratios and control factors
- ETS coupling efficiency, j≈E: j≈E = ≈E/E = (E-L)/E = 1-L/E
- LEAK control ratio, L/E
- Excess E-P capacity factor, jExP: jExp = (E-P)/E = 1-P/E
- OXPHOS control ratio, P/E
- OXPHOS coupling efficiency, j≈P: j≈P = ≈P/P = (P-L)/P = 1-L/P
- L/P coupling control ratio, L/P
- Respiratory acceptor control ratio, RCR: RCR = State 4/State 3
- netOXPHOS control ratio, ≈P/E: ≈P/E = (P-L)/E
- Uncoupling control ratio, UCR: UCR = E/R
Conclusions
- With a perspective to extend the present recommendations on coupling control (part 1) to pathway control of mitochondrial respiration (part 2), substrate-uncoupler-inhibitor-titration (SUIT) protocols, and harmonization of experimental procedures, MITOEAGLE will be a gateway and milestone to better diagnose mitochondrial respiratory defects which are linked to various age-related health risks, including cardiovascular and degenerative diseases, such as type 2 diabetes, neurodegenerative diseases (Alzheimer’s, Parkinson’s, Huntington’s), and several types of cancer.
List of selected terms and symbols
- Basal respiration
- Cell respiration
- Coupling control factor, CCF
- Coupling control ratio, CCR
- Coupled respiration
- Dyscoupled respiration
- Intact cells, ce
- Isolated mitochondria, imt
- Mitochondria, mt (Greek mitos: thread; chondros: granule) are small structures within cells, which function in cell respiration as powerhouses or batteries. Mitochondria belong to the bioblasts of Richard Altmann (1894). Abbreviation: mt, as generally used in mtDNA. Singular: mitochondrion (bioblast); plural: mitochondria (bioblasts).
- Mitochondrial inner membrane, mt-im
- Mitochondrial outer membrane, mt-om
- Mitochondrial matrix, mt-matrix
- Mitochondrial membrane potential, mtMP, Δψmt
- Mitochondrial preparations, mtprep are isolated mitochondria (imt), tissue homogenate (thom), mechanically or chemically permeabilized tissue (permeabilized fibres, pfi) or permeabilized cells (pce). In these preparations the cell membranes are either removed (imt and smtp) or mechanically (thom) and chemically permeabilized (pfi), while the mitochondrial functional integrity and to a large extent the mt-structure is maintained.
- Mitochondrial respiration
- Noncoupled respiration
- Oligomycin, Omy
- Permeabilized tissue or cells, pti, pce
- Phosphorylation system
- Proton leak
- Proton pump
- Proton slip
- Protonmotive force, Δpmt
- Resting metabolic rate
- Tissue homogenate, thom
- Uncoupler, U
References
- Altmann R (1894) Die Elementarorganismen und ihre Beziehungen zu den Zellen. Zweite vermehrte Auflage. Verlag Von Veit & Comp, Leipzig:160 pp, 34 Tafeln. - »Bioblast link«
- Chance B, Williams GR (1955) Respiratory enzymes in oxidative phosphorylation. I. Kinetics of oxygen utilization. J Biol Chem 217:383-93. - »Bioblast link«
- Chance B, Williams GR (1955) Respiratory enzymes in oxidative phosphorylation. III. The steady state. J Biol Chem 217:409-27. - »Bioblast link«
- Chance B, Williams GR (1956) The respiratory chain and oxidative phosphorylation. Adv Enzymol17:65-134. - »Bioblast link«
- Cohen ER, Cvitas T, Frey JG, Holmström B, Kuchitsu K, Marquardt R, Mills I, Pavese F, Quack M, Stohner J, Strauss HL, Takami M, Thor HL (2008) Quantities, Units and Symbols in Physical Chemistry, IUPAC Green Book, 3rd Edition, 2nd Printing, IUPAC & RSC Publishing, Cambridge. - »Bioblast link«
- Estabrook R (1967) Mitochondrial respiratory control and the polarographic measurement of ADP:O ratios. Meth Enzymol 10:41-7. - »Bioblast link«
- Galluzzi L, Vitale I, Abrams JM, Alnemri ES, Baehrecke EH, Blagosklonny MV, Dawson TM, Dawson VL, El-Deiry WS, Fulda S, Gottlieb E, Green DR, Hengartner MO, Kepp O, Knight RA, Kumar S, Lipton SA, Lu X, Madeo F, Malorni W, Mehlen P, Nuñez G, Peter ME, Piacentini M, Rubinsztein DC, Shi Y, Simon HU, Vandenabeele P, White E, Yuan J, Zhivotovsky B, Melino G, Kroemer G (2012) Molecular definitions of cell death subroutines: recommendations of the Nomenclature Committee on Cell Death 2012. Cell Death Differ 19:107-20. - »Bioblast link«
- Garlid KD, Semrad C, Zinchenko V (1993) Does redox slip contribute significantly to mitochondrial respiration? In: Schuster S, Rigoulet M, Ouhabi R, Mazat J-P (eds) Modern trends in biothermokinetics. Plenum Press, New York, London:287-93. - »Bioblast link«
- Gnaiger E (1993) Nonequilibrium thermodynamics of energy transformations. Pure Appl Chem 65:1983-2002. - »Bioblast link«
- Gnaiger E (1993) Efficiency and power strategies under hypoxia. Is low efficiency at high glycolytic ATP production a paradox? In: Surviving Hypoxia: Mechanisms of Control and Adaptation. Hochachka PW, Lutz PL, Sick T, Rosenthal M, Van den Thillart G (eds) CRC Press, Boca Raton, Ann Arbor, London, Tokyo:77-109. - »Bioblast link«
- Gnaiger E (2009) Capacity of oxidative phosphorylation in human skeletal muscle. New perspectives of mitochondrial physiology. Int J Biochem Cell Biol 41:1837-45. - »Bioblast link«
- Gnaiger E (2014) Mitochondrial pathways and respiratory control. An introduction to OXPHOS analysis. 4th ed. Mitochondr Physiol Network 19.12. OROBOROS MiPNet Publications, Innsbruck:80 pp. - »Bioblast link«
- Gnaiger E, Méndez G, Hand SC (2000) High phosphorylation efficiency and depression of uncoupled respiration in mitochondria under hypoxia. Proc Natl Acad Sci U S A 97:11080-5. - »Bioblast link«
- International Union of Biochemistry and Molecular Biology: Recommendations for terminology and databases for biochemical thermodynamics. - »Open Access«
- Hatefi Y, Haavik AG, Fowler LR, Griffiths DE (1962) Studies on the electron transfer system. XLII. Reconstitution of the electron transfer system. J Biol Chem 237:2661-9. - »Bioblast link«
- International Union of Biochemistry (1981) Symbolism and terminology in enzyme kinetics. - »Open Access«
- Lemieux H, Blier PU, Gnaiger E (2017) Remodeling pathway control of mitochondrial respiratory capacity by temperature in mouse heart: electron flow through the Q-junction in permeabilized fibers. Sci Rep 7:2840, DOI:10.1038/s41598-017-02789-8. - »Bioblast link«
- Miller GA (1991) The science of words. Scientific American Library New York:276 pp. - »Bioblast link«
- Mitchell P (1961) Coupling of phosphorylation to electron and hydrogen transfer by a chemi-osmotic type of mechanism. Nature 191:144-8. - »Bioblast link«
- Mitchell P (1966) Chemiosmotic coupling in oxidative and photosynthetic phosphorylation. Glynn Research Ltd, Bodmin:192 pp. - The Grey Book 1.
- Mitchell P (1968) Chemiosmotic coupling and energy transduction. Glynn Research Ltd, Bodmin:111 pp. - The Grey Book 2.
- Mitchell P, Moyle J (1967) Respiration-driven proton translocation in rat liver mitochondria. Biochem J 105:1147-62. - »Bioblast link«
Mitochondrial respiratory control: pathway states in mt-preparations
- MITOEAGLE recommendations Part 2.
- The mitochondrial respiratory system
- Substrates and inhibitors
- Switch to pathway-related nomenclature instead of enzyme-linked terminology (N/NS/S versus CI/CI+II/CII)
Action
- » WG1 Action - WG1 MITOEAGLE protocols, terminology, documentation: Standard operating procedures and user requirement document: Protocols, terminology, documentation
- » WG1 Project application
- » 2017-07 MiPschool Obergurgl 2017
- » 2017-03 MITOEAGLE Barcelona 2017
- » 2016-11 MITOEAGLE 2016 Verona IT